Mitochondria are organelles found within cells responsible for energy (ATP) production. Such is achieved via conversion of food (carbohydrates, fats, proteins) through oxidation and enzymatic reactions (Reisner & Reisner, 2017). As such, it is paramount said enzymes are present and in adequate concentrations, which is achieved by appropriate levels of key precursors; zinc (Zn) and copper (Cu). Adequate, and balanced, levels of said micronutrients provides a platform and opportunity for increased energy levels, health, and homeostasis. Disruption in either consumption, digestion, or absorption can become an impediment to said biomarkers in addition to compromised Fe absorption. As such, the following will explore the function of Zn and Cu, their relationship to mitochondrial function, Fe, and energy production.
Zn is a micronutrient involved in the synthesis and function of over 300 enzymes and proteins, deeming its presence vital (Young et al., 2014). Said mineral is absorbed primarily in the proximal small intestine and is believed to be liberated from the foods it is bound to with the help of a highly acidic medium (i.e., stomach via hydrochloric acid) (Gropper, Smith, & Carr, 2018). Once digested, absorption occurs via carrier-mediated transport systems and paracellular diffusion (Grooper et al., 2018).
Carrier-mediated transport is achieved through Zrt and Irt-like proteins, known as ZIP4 carriers, and is expressed/activated when 7-9 mg of Zn are present in the lumen (Gropper et al., 2018; Lu, Haragopal, Slepchenko, Stork, & Li, 2016). When Zn levels rise to 20 mg or higher in the intestine, absorption exceeds the capacity of ZIP4 transporters. Once Zn is within the enterocyte (intestinal cell), movement towards the blood stream is facilitated by zinc 1 transporters (ZnT1) (Gropper et al., 2018). At such a point, paracellular diffusion (movement between intestinal cells) initiates to continue Zn absorption (Gropper et al., 2018). Thus, any compromise in acidity, ZIP4/ZnT1 expression, or paracellular diffusion will limit Zn concentrations in the blood.
Within the mitochondria, Zn serves as a cofactor in the synthesis of a protein known as Zim17, which facilitates protein folding and importation of proteins (Pierrel, Cobine, & Winge, 2007). Furthermore, Zn serves in mitochondrial metabolic functions to include alcohol oxidation, and leucine (an essential amino acid involved in protein formation) synthesis. Furthermore, Zn is required for the proper development, structure, and function of cytochrome c oxidase; an enzyme in mitochondria (among others) that contributes to the production of ATP via hydrogen ion transport and aggregation (Pierrel et al., 2007). Having considered the absorption, transport, and mitochondrial function of Zn, the following will explore Cu in addition to its absorption, transport, function within said organelle.
Cu is a micronutrient involved in several biochemical and physiological processes throughout the body. Said mineral is found in food that is bound to other substances consumed in the diet, namely amino acids from protein (Gropper et al., 2018). As such, digestion is required to liberate and reduce Cu in the presence of acid (i.e., hydrochloric acid in the stomach) pepsin, and other proteolytic enzymes (Gropper et al., 2018). Once reduced, Cu1+ is transported into enterocytes (intestinal cells) via transporters known as copper transporter 1 (Ctr1); a carrier expressed by the presence of Cu1+. Cu is also transported by divalent mineral transporter (DMT1); a unique carrier in that DMT1 not only transports Cu1+ but also hydrogen ions (i.e., H+) (Gropper et al., 2018).
Cu functions in the oxidation of Fe, collagen and elastin cross-linking, synthesis of norepinephrine, melanin/pigment production, blood clotting, and immune function (Gropper et al., 2018). Within the context of mitochondrial function, Cu is involved in the synthesis of superoxide dismutase (i.e., an antioxidant) and cytochrome c oxidase (i.e., involved in ATP production) (Gropper et al., 2018). Superoxide dismutase (SOD1) requires both Zn and Cu to function properly; both Zn and Cu are bound to the enzyme by an imidazole group with Cu residing on the active site of SOD1 (Ruiz et al., 2014).
Such an enzyme is relevant as it controls the buildup of superoxide; a potentially harmful/toxic by-product of ATP production in the mitochondria. Cu deficiency down regulates SOD1 activity, allowing the buildup of superoxide; a potent free radical that damage the phospholipid components of cell membranes (Gropper et al., 2018). Cytochrome c oxidase (CCO) is an enzyme within the mitochondria responsible for transporting and aggregating H+ to form ATP; its structure and function requires Cu to receive and transfer electrons (Gropper et al., 2018). As such, the presence of Cu in the diet, and successful absorption/transport, is essential for optimal functioning of both SOD1 and CCO. Having considered the influence of Cu and Zn upon mitochondrial processes, the following will explore the relationship between Fe and Zn.
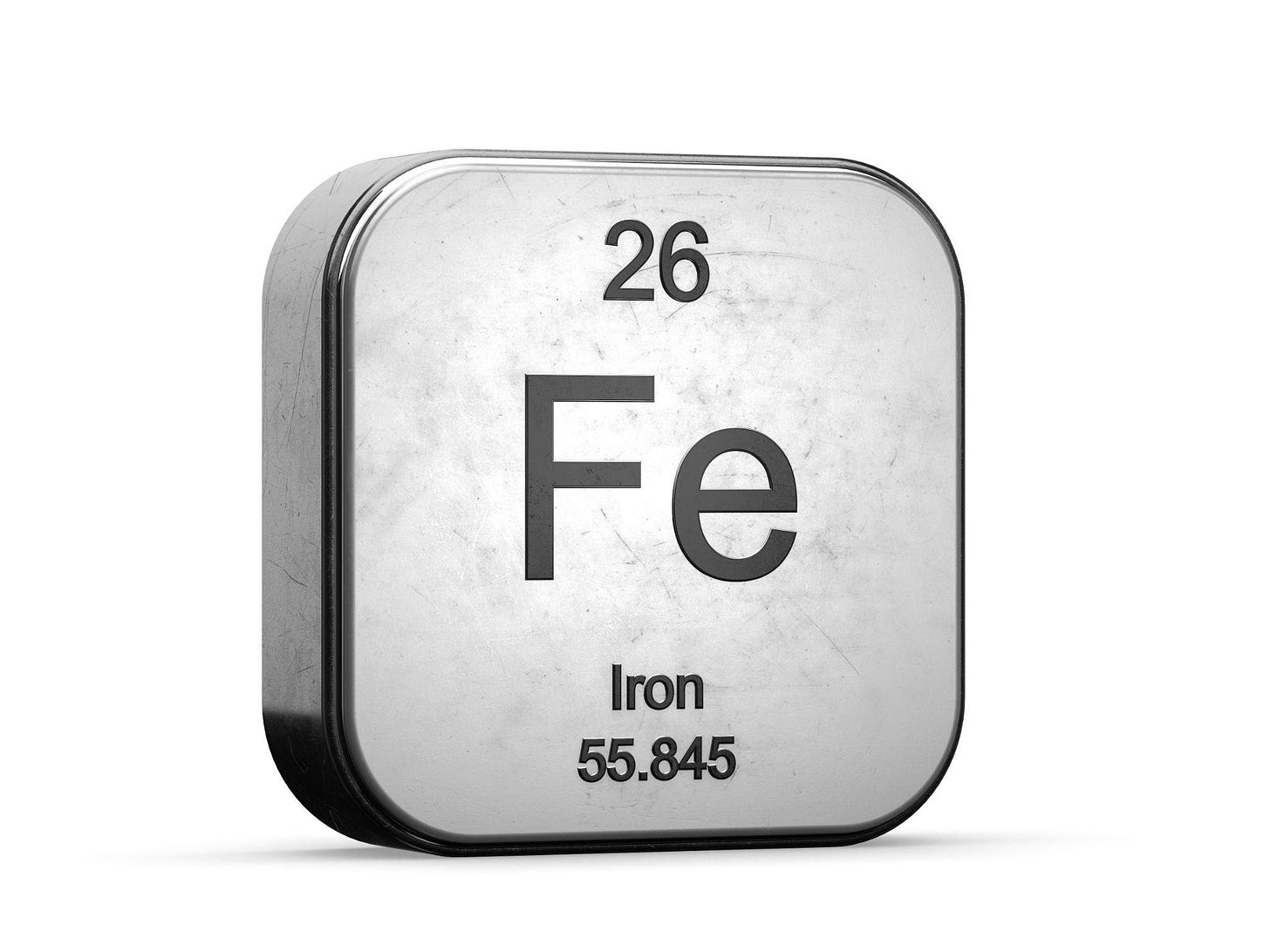
Fe is a micronutrient that helps form several proteins in addition to several dozen enzymes, all involved in a multitude of physiological processes (Gropper et al., 2018). Such functions of Fe include, but are not limited to, DNA synthesis, carbohydrate/amino acid metabolism, thyroid hormone synthesis, destruction of bacteria/viruses/microbes, electron transport (within mitochondria), synthesis of hemoglobin/myoglobin, antioxidant function, and nitric oxide synthesis (Gropper et al., 2018). As such, adequate consumption, absorption, and transport of Fe is essential to maintain such processes.
Generally, Fe is found within food as heme (i.e., animal sources) and non-heme (i.e., plant sources). For each form, high acidity (i.e., hydrochloric acid) and proteases (i.e., enzymes that hydrolyze proteins) among other substances are required for the successful digestion of each (Gropper et al., 2018). Furthermore, carrier proteins are necessary for entrance into the enterocyte; heme-bound Fe uses the heme-carrier protein (HCP) as well as the proton-coupled folate transporter (PCFT) while non-heme Fe uses a divalent cation transporter 1 (DCT). Finally, movement across the basolateral membrane requires the use of ferroportin, and blood transport requires transferrin (Gropper et al., 2018). Although such transporters work efficiently in a healthy individual, Fe absorption can become inhibited by other micronutrients. The following will consider the same.
Gropper et al. (2018) indicated that some micronutrients can hinder the absorption of Fe such as polyphenols, oxalic acid, phytic acid, phosvitan, and divalent cations such as manganese, calcium, and Zn. Zn for example, although necessary for a myriad of processes, has the capacity to inhibit non-heme Fe absorption by as high as 50%. However, Gropper et al. (2018) could not identify the mechanism of action. Consequently, recommendations of Zn consumption are limited to approximately 11 mg/day for males and 8 mg/day for females with a tolerable upper intake of 40 mg/day (Gropper et al., 2018). Levels above the safe upper limit may contribute to compromised Fe absorption.
In conclusion, micronutrients such as Zn and Cu are imperative to optimal mitochondrial function while Fe plays a role in several processes discussed in previous sections. Although such micronutrients are essential for homeostasis, it is also relevant to consider said minerals within the context of a system; one must consume adequate amounts of foods rich in the aforementioned nutrients, the body must exhibit proper digestion to include adequate amounts of acidity and proteases, in addition to consumption of balanced levels of nutrients in the diet so as not to down-regulate absorption (i.e., lowered Fe absorption from excessive Zn consumption). If one abides by such an approach, the likelihood of micronutrient deficiencies are minimized, providing opportunity for improved health, performance, and longevity.
References
Gropper, S. S., Smith, J. L., & Carr, T. P. (2018). Advanced nutrition and human metabolism (7thed.). Boston, MA: Cengage Learning.
Lu, Q., Haragopal, H., Slepchenko, K. G., Stork, S., & Li, Y. V. (2016). Intracellular zinc distribution in mitochondria, ER and the golgi apparatus. International Journal of Physiology, Pathophysiology, and Pharmacology, 8(1), 35-43.
Pierrel, F., Cobine, P. A., & Winge, D. (2007). Metal ion availability in mitochondria. Biometals, 20(3), 675-682.
Reisner, E. G., & Reisner, H. M. (2017). An introduction to human disease: Pathology and pathophysiology correlations (10thed.). Burlington, MA: Jones & Bartlett Learning.
Ruiz, L. M., Jenson, E. J., Bustos, R. I., Arguelloa, G., Gutierrez-Garcia, R., Gozalez, M.,…Elorza, A., A. (2014). Adaptive responses of mitochondria to mild copper deprivation involve changes in morphology, OXPHOS remodeling and bioenergetics. Journal of Cellular Physiology, 229(5), 607-619.
Young, G. P., Mortimer, E. K., Gopalasmy, G. L., Alpers, D. H., Binder, H. J., Manary, M. J., … Brewer, T. G. (2014). Zinc deficiency in children with environmental enteropathy – Development of new strategies: Report from an expert workshop. The American Journal of Clinical Nutrition, 100(4), 1198-1207.
-Michael McIsaac