The human body communicates, interacts, and coordinates with itself and the external environment through a dense network of electrochemical conduits, colloquially known as the nerves or nervous system (NS) (Kenney, Wilmore, & Costill, 2012). Virtually all tissues, organs, motor, and sensory systems are fully integrated with the NS; like a conductor in an orchestra, the NS guides the organism’s internal and external functions in a highly coordinated, purposeful, and efficacious fashion.
However, the NS (to include the neuromuscular system), like other systems in the human body, can experience vulnerability and compromise, ultimately affecting homeostasis. Considering the interconnectedness of the NMS with the body and environment, aberrations in its function, by default, can induce systemic affects throughout the organism. One such manifestation of a dysregulated NMS is muscle weakness and fatigue often associated with underlying micronutrient imbalances/deficiencies (Gropper, Smith, & Carr, 2018).
As a means of appreciating the NMS and its relationship to nutrition, the following will explore NMS regulation/dysregulation, causes of the same, the relationship of the NMS to micronutrient deficiencies (with a particular focus on electrolytes), and solutions to help mitigate and track said deficiencies.
The NS is composed and organized into a hierarchy beginning with the central nervous system (CNS) and the peripheral nervous system (PNS). The CNS is comprised of the spinal cord and brain, while the PNF is composed of the sensory nerves (i.e., afferent) and the motor nerves (i.e., efferent) (Kenney et al., 2012; Reisner & Reisner, 2017). The afferent nerves are responsible for providing information to the CNS regarding physiological and biochemical activities within the body in addition to information regarding the environment outside of the organism.
The efferent nerves execute commands from the CNS based on information gained from the afferent system directing its signals towards organs and tissues throughout the body (Kenney et al., 2012). The impetus behind such signaling is made possible by way of electrochemical reactions along, and within, the neurons of the NS (Reisner & Reisner, 2017). Such a process is integral to the successful transfer of impulses, and ultimately, information throughout the body. The following will explore the same in greater detail.
Neurons are the most basic functional element of the NS composed of the axon, dendrites, and cell body (Kenney et al., 2012). The cell body contains fingerlike projections called dendrites, which receive impulses from other neurons. The axon is the impulse transmitter of the neuron conducting its signals from the cell body towards the dendrites, which contains neurotransmitters that are released and communicate with another neuron or cell (Kenney et al., 2012).
Integral to the process as a whole is the successful generation of the impulse itself. Such an event is made possible by overcoming the resting membrane potential (RMP) of the neuron. RMP is defined as the difference in electrical charge of the outside of the neuron compared to the inside of the neuron (Kenney et al., 2012).
In a resting state of the neuron, the intracellular charge is -70 mV with an overall negative charge intracellularly, and a positive charge extracellularly (Kenney et al., 2012). Such a potential is maintained by regulating the concentration of potassium ions (K+) intracellularly and concentrations of sodium ions (Na+) extracellularly, otherwise known as polarization (Kenney et al., 2012).
Said balance of ions is regulated by a semipermeable membrane allowing the influx of K+over Na+via ion channels in addition to a K+/Na+ pump (i.e., K+-Na+ATPase), which transports 2 K+ inside the cell and 3 Na+ outside of the cell during each K+-Na+ATPase cycle (Kenney et al., 2012). When a sufficient stimulus (i.e., impulses from another neuron or chemical/temperature/pressure) overcomes RMP/polarized state of the neuron, full neuronal depolarization occurs; a seminal event, which allows impulse transmission along the entirety of the neuron (i.e., action potential).
Specifically, depolarization is initiated by the aforementioned ion gates opening allowing Na+to rush inwardly. If enough ion gates open from a sufficient stimulus to create a substantial and rapid depolarized state (i.e., an action potential) from -70mV to -50 to -55 mV (the threshold), a nerve impulse will propagate along the entire length of the neuron. (Kenney et al., 2012). Having briefly considered the NS and its relationship to electrolytes (i.e., Na+and K+), the following will consider the NS and its relationship to muscle function, commonly known as the NMS.
Once a successful action potential has reached the synapse of a dendrite, neurotransmitters (i.e., acetylcholine) are released between the synaptic cleft rapidly contacting the muscle fiber surface (i.e., plasmalemma) (Kenney et al., 2012). A second action potential is then created along, and within, the muscle fiber via T-tubules where calcium (Ca2+)ions (a third electrolyte) are released from their storage sites (i.e., sarcoplasmic reticulum) into the sarcoplasm (i.e., the cytoplasm of a muscle fiber) (Kenney et al., 2012).
Once such a process begins, Ca2+)facilitates the contraction of actin/myosin filaments within sarcomeres; the basic contractile units of muscle. Such a process, known as the excitation-contraction coupling requires the influx of Ca2+ to expose the active sites (Ca2+binds to troponin, enabling troponin to lift tropomyosin off of actin allowing actin/myosin contact) where actin and myosin mechanically interact with each other and ultimately, contract (Kenney et al., 2012).
Although the presence of Ca2+ is central to the actin/myosin engagement, such an electrolyte when left unchecked, can induce excessive contractions along the sarcomere. The following will consider how such an ion is regulated.
Although Ca2+ is important in muscle contraction, an overabundance of said electrolyte can initiate excessive myosin/actin activity. As such, a fourth electrolyte, magnesium (Mg2+), competes for placement on troponin, thereby down-regulating the frequency of contractions within the sarcomere (Garrison, Allan, Sekhon, Musini, & Khan, 2012; Potter, Robertson, & Johnson, 1981). As evidenced in the aforementioned sections, a well-functioning NMS is made possible by several mechanisms working together in a highly orchestrated and coordinated fashion.
Most importantly, the presence of Na+, K+, Ca2+, and Mg+is foundational to the optimal regulation of RMP, initiation of the action potential, and contraction of muscle. As such aberrations in said micronutrient availability can manifest as neuromuscular dysfunctions. Thus, the following will explore underlying physiological mechanisms and causes behind a dysregulated NMS, with emphasis on muscular fatigue (paresis) and cardiac dysfunction (Balci, Koksal, Kose, Armagan, Ozdemir, Inal, & Oner, 2013).
Electrolyte deficiencies can emanate from multiple sources to include inadequate nutrition, excessive physical activity, metabolic disorders (Balci et al., 2013; Cogswell et al., 2012; Kenney et al., 2012). For example, in a study conducted between 2003-2008 among the US population, Cogswell et al. (2012) determined that most American adults overconsumed Na+ while less than 2% of the population consumed adequate amounts of K+. Consequently, such a deficiency/imbalance has contributed to NMS dysregulation to include hypertension (Cogswell et al., 2012).
Other research has indicated that excessive physical activity (i.e., sweating) can compromise electrolyte levels (Mao, Chen, & Ko, 2001). Since the body maintains a constant internal temperature of 37 degrees Celsius, circumstances (i.e., hot environment or increased activity) may arise in which temperature is regulated by heat dissipation via sweating (Mao, Chen, & Ko, 2001; Popovic, Momenroodaki, & Scheeler, 2014). Sweating not only releases water; abundant levels of Na+, Chloride (Cl–), K+, and Ca2+ are also present (Mao et al., 2001).
Since such electrolytes are responsible for neuromuscular excitability, membrane permeability, and impulse transmission, severe losses from sweating can induce tremors, cardiac arrhythmia, and paresis (Mao et al., 2001). Finally, electrolyte deficiencies can come from metabolic disorders such as chronic kidney disease, defined as a gradual loss of kidney function (Dhondup & Qian, 2017). Since the kidneys play a central role in acid-base regulation and electrolyte balance, disruption in said system can lead to electrolyte losses (Dhondup & Qian, 2017).
Among diabetics, for example, long standing diabetes can develop progressive renal damage from insulin resistance (Lecube, Baena-Fustegueras, Fort, Pelegri, Hernandez, & Simo, 2012). Under normal conditions, the kidneys reabsorb Mg2+when stimulated by insulin. However, insulin resistance, a central characteristic of type 2 diabetes, down regulates the kidneys’ capacity resorb Mg2+. Such an event can dramatically lower Mg2+levels (Lecube et al., 2012). Having considered NMS regulation, dysregulation, and causes of the same, the following will explore a nutritional treatment plan that minimizes foods which worsen the condition, while introducing foods and supplements, where indicated, that ameliorate neuromuscular and cardiac health.
Na+is a micronutrient generally found in abundance within the human diet; 75% of daily consumption is derived from processed foods (Gropper et al., 2018). Furthermore, the average North American consumes approximately 3371 mg/day of Na+; a level that exceeds the upper intake (2300 mg/day) in excess of 1000 mg, which can be associated with hypertension (Gropper et al., 2018). Physiologically, hypertension occurs from a high concentration of Na+outside of the cell; higher extracellular concentration gradients, compared to intracellular levels, increases the rate of depolarization (Seyama & Irisawa, 1967).
Although the general theme of this author’s paper is to address micronutrient deficiencies and their relationship to NMS dysfunction and paresis, Na+ is an electrolyte with significant presence in the food system due to its capacity to enhance shelf life and taste of foods (Albarracin, Sanchez, Grau, & Barat, 2011). As such, one intervention to lower Na+ to optimal levels might include a reduction in processed foods (i.e., canned foods, processed carbohydrates, processed meats).
Caution should be taken to make sure levels remain optimal; dramatic reductions in processed food would mean dietary Na+would come from whole food sources (i.e., meats, poultry, vegetables) of which only 10% of daily Na+requirements are derived from (Gropper et al., 2018). Additionally, vigorous exercise/sweating (as explained in the previous section) can induce electrolyte losses to include Na+. As a preventative measure, one should monitor signs and symptoms of Na+deficiency to include cramps, nausea, vomiting, and dizziness when reducing Na+rich foods from the diet (Gropper et al., 2018).
Solutions could include introducing Na+over foods in the form of table salt (i.e., ½ to 1 teaspoon = up to 2300 mg). If signs and symptoms remain, a periodic 24-hour urinary sodium tests can be performed; if levels are below 5-10 mmol/L, Na+levels are considered deficient (Gropper et al., 2018). If deficiency is identified, slowly increasing Na+ levels might be indicated. Having noted excessive Na+ consumption as a potential impediment to optimal NMS/cardiac function, the following will explore nutrients and foods which can help optimize the same.
In previous sections, K+ was described as an electrolyte involved in maintenance of RMP and depolarization; precursors of the action potential (nerve impulse) and muscle contraction in both skeletal and cardiac tissue (Kenney et al., 2012). Maintenance of the RMP includes regulating 2 K+intracellularly to every 3 Na+extracellularly during a nerve’s resting state (maintained via the K+/Na+ pump) (Kenney et al., 2012).
As was the case with excessive Na+, lower K+levels (hypokalemia) also disrupt the concentration gradient; repolarization involves K+migrating back into the cell to re-establish the RMP. However, when less K+ is available (hypokalemia), it takes longer to restore the RMP (known as the refractory period) (Kenney et al., 2012). Consequently, much larger stimuli are required to initiate a second action potential during this time period, if at all (Kenney et al., 2012).
Signs and symptoms of deficiency include muscle weakness, nausea, vomiting, obstipation (severe constipation), polyuria (excessive urination), polydipsia (excessive thirst), cardiac arrhythmias, and paresis (Gropper et al., 2018; Jankovic, 2018). Left untreated, even moderate hypokalemia increases risk of mortality in those with cardiovascular disease (Gennari, 1998).
Serum/plasma blood tests can help unmask deficiencies; if K+levels are below 3.6 mmol/L, hypokalemia is present. Levels below 2.5 mmol/L can induce muscle necrosis, while levels below 2.0 mmol/L can cause respiratory dysfunction and ascending paralysis (Gennari, 1998). Having considered the function, pathophysiology, and testing of K+, the following will outline food sources and supplementation of said micronutrient.
K+ can be found in a larger spectrum of foods to include meat, fruits, and vegetables such as avocados, leafy green vegetables, nuts, seeds, mushrooms, and asparagus (Gropper et al., 2018; Lanham-New, Lambert, & Frasetto, 2012). Such foods are also beneficial because there is often a presence of other essential and beneficial micronutrients (Kovesdy et al., 2017). The current adequate intake recommendations for adults is approximately 4700 mg/day (Gropper et al., 2018). However, both adult males and females are not reaching said recommendations (Kovesdy et al., 2017).
If signs and symptoms of hypokalemia persist (supported by K+ blood measures; <3.0 mEq/L = deficiency), despite ingestion of K+-rich foods, supplementation might be indicated (Gropper et al., 2018). If so, Gropper et al. (2018) suggested use of enteric-coated capsules (to help reduce gut irritation) in the form of a salt (i.e., potassium gluconate, citrate, phosphate, bicarbonate) and in multiple/smaller doses throughout the day.
Supplementation should be undertaken with caution due to the concentration of K+compared to food sources; high levels of said micronutrient can cause hyperkalemia characterized by muscle weakness, twitching, cramping, paralysis, and death. As such, periodic measures of K+levels ( >3.5 mEq/L is normal), maintaining a food diary, and monitoring of signs and symptoms of hyperkalemia is recommended (Gropper et al., 2018).
Mg2+ is the second most abundant electrolyte after K+involved in excess of 300 biochemical reactions in the body (Gropper et al., 2018). As was mentioned in previous sections, Mg2+ helps control the rate and degree of muscle contraction/force production by competitively binding to troponin sites over Ca2+within the sarcomere (Garrison et al., 2012). Such balance with Ca2+ helps ensure smooth force production and minimization of cramping (Garrison et al., 2012).
Signs of deficiency (hypomagnesemia) can include muscle weakness, tremors, twitching, cramps and tetany which can be corroborated by plasma concentrations of Mg2+of less than 1.2 mg/dL (Gropper et al., 2018). As such, it is imperative that adequate levels of Mg2+ are maintained throughout the body and can be achieved via food sources such as (but not limited to) meat, seafood, green leafy vegetables, nuts, and seeds (Wilcock & Twycross, 2013).
The Daily Value for Mg2+ is set at 400 mg, however, approximately two thirds of the population are not reaching said target (Schwalfenberg & Genuis, 2017). If food sources cannot improve signs, symptoms, and plasma levels of Mg2+, supplementation might be indicated in the form of 300-600 mg/day for mild deficiency defined as plasma levels between 1.1 and 1.4 mg/dL (Gropper et al., 2018).
Although toxicity is unlikely to be reached via food sources, supplemental forms of Mg2+in excess may induce symptoms to include diarrhea, nausea, vomiting, muscle weakness, hypotension, apnea, paralysis, and cardiac/respiratory failure (Gropper et al., 2018). As such, it is imperative to be cognizant of signs and symptoms of acute or severe toxicity Mg2+. Periodic plasma measures during supplementation, and tracking of Mg2+from food, might be indicated during said process to adjust to the appropriate dosage.
Ca2+ is the final electrolyte responsible for inducing actin/myosin crossover upon its contact with troponin within the sarcomere, as covered previously. Furthermore, Ca2+increases the rate and degree of muscle contraction as it competes against Mg2+ for placement on troponin (Garrison et al., 2012). Although Ca2+ directly interacts with the sarcomere, it also serves to control pre-synaptic events related to depolarization. For example, voltage-gated Na+channels are responsible for directing influx of said ion during depolarization.
With enough adequate stimulus (i.e., impulses from another neuron or chemical/temperature/pressure) voltage-gated Na+channels are opened to move the RMP from -70 mV to -50 mV (i.e., the threshold) to induce an action potential (Kenney et al., 2012). Interestingly, extracellular Ca2+ levels can affect the degree of stimulus required. Specifically, Han, Trinidad, & Shi (2015) stated that lower extracellular Ca2+(hypocalcemia) levels shifted the RMP to the left, enabling a smaller stimulus to reach threshold thereby increasing neuronal excitability. Ca2+ also directly affects K+ ion activity by inhibiting Ca2+ activated K+channels (KCA channel) (Han et al., 2015).
Such channels are responsible for K+efflux during depolarization and are responsible for the hyperpolarized state (relative refractory period) that occurs shortly thereafter. Hypocalcemia decreases the activity of such KCA channels minimizing the degree of K+efflux and hyperpolarization (Han et al., 2015). Ultimately, such a scenario increases neuronal excitability and firing rates. Signs and symptoms of hypocalcemia (in addition to neuronal excitability and firing rates) include muscles that fail to relax, severe muscle cramps, respiratory dysfunction, and in severe cases, death (Gropper et al., 2018).
Testing Ca2+ blood levels would be a logical route to determine if an individual was experiencing hypocalcemia. However, because of tight Ca2+ regulation, no routine testing measures have been effective except for advanced diseased states (Gropper et al., 2018). Rather, tracking and monitoring of food intake serves as an acceptable measure. Having considered the function, pathophysiology, and testing of Ca2+,the following will outline food sources, supplementation, and signs of Ca2+toxicity.
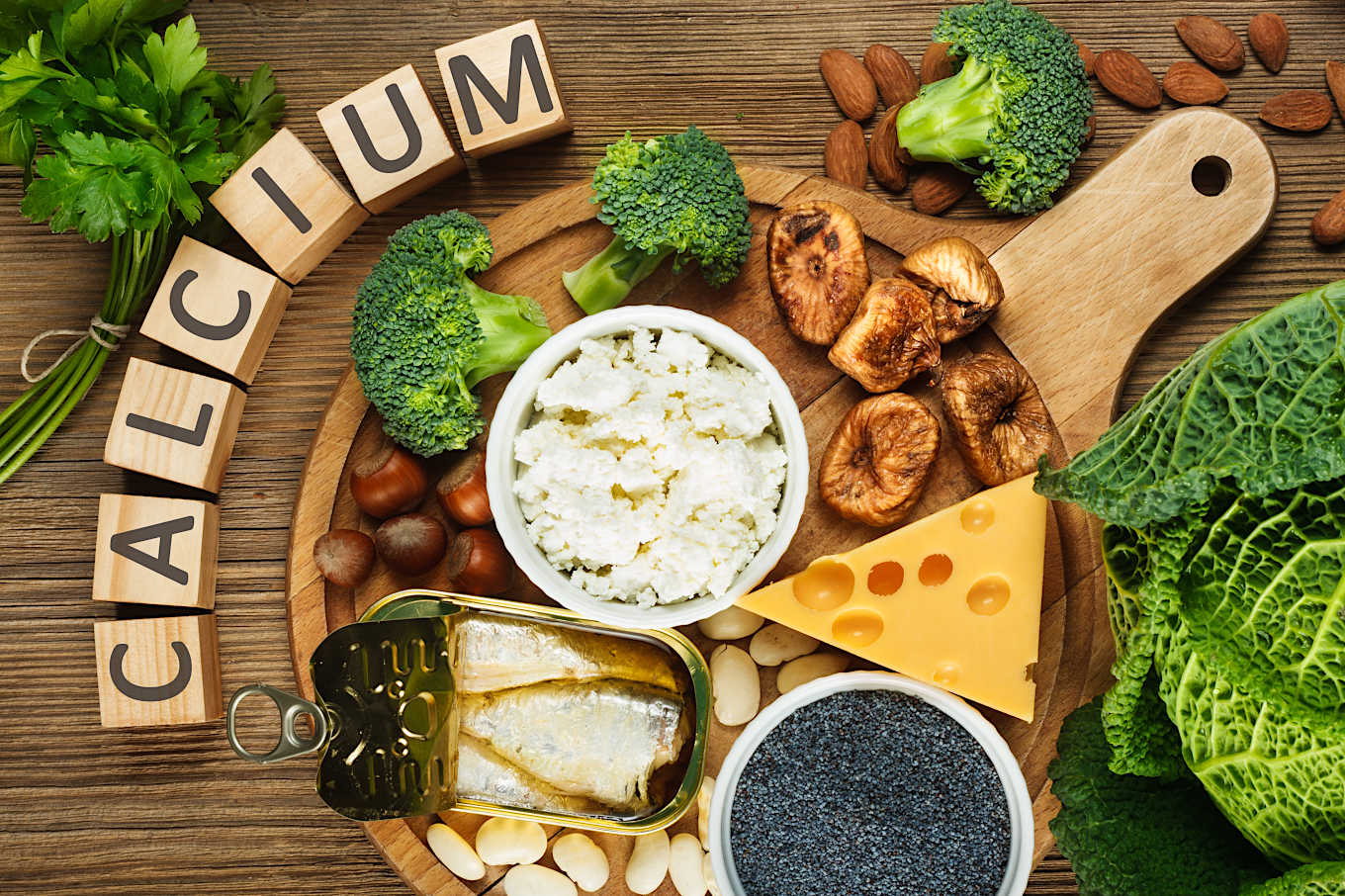
Ca2+ can be found in foods such as dairy (i.e., milk, cheese, yogurt), seafood (i.e., salmon, sardines), and some vegetables (i.e., turnip, mustard greens, broccoli, kale) (Gropper et al., 2018). Due to the roles mentioned in the previous section, it is paramount that individuals consume adequate levels each day. The Recommended Dietary Allowance for adults ranges from 1000-1200 mg/day (Gropper et al., 2018). If an individual cannot consume enough food to reach levels/has a down-regulated calcium transporter 1 (occurs with aging), supplementation might be indicated (Gropper et al., 2018).
Multiple forms of Ca2+supplements exist; however, calcium citrate is a form that is the most digestible, especially in the presence of vitamin D, as it does not require high acidities in the gut. (Gropper et a., 2018). Furthermore, the Tolerable Upper Intake for Ca2+ is 2000-2500 mg/day. As such, awareness of dietary Ca2+intake and supplementation totals are warranted to avoid hypercalcemia; a condition characterized and associated with excessive Ca2+consumption (i.e., 4 g/day), arrhythmias, bradycardia, coma, and death (Gropper et al., 2018).
In conclusion, neuromuscular regulation is a highly orchestrated event demanding a balanced participation of electrolytes such as Na+, K+, Mg2+, and Ca2+to conduct impulses, and information, throughout the human movement system. However, the NMS, like other systems, can experience vulnerability and compromise. Such dysregulation has been observed by electrolyte deficiencies to include muscular fatigue, twitching, cardiac arrhythmias, paresis, and even death. However, awareness of signs and symptoms of each electrolyte deficiency, in addition to tracking food and blood biomarkers, can allow the opportunity for intervention using a food-as-medicine paradigm, and supplementation of electrolytes when warranted. Ultimately, such pragmatic steps should help liberate individuals from muscle weakness and cardiac dysfunction leading to improved health, performance, and quality of life.
References
Albarracin, W., Sanchez, I., Grau, R., & Barat, J. M. (2011). Salt in food processing; Usage and reduction: A review. International Journal of Food Science and Technology, 46(7), 1329-1336.
Balci, A. K., Koksal, O., Kose, A., Armagan, Ozdemir, E., Inal, T., & Oner, N. (2013). General characteristics of patients with electrolyte imbalance admitted to emergency department. World Journal of Emergency Medicine, 4(2), 113-116.
Cogswell, M. E., Zhang, Z., Carriquiry, A. L., Gunn, J. P., Kulina, E. V., Saydah, S. H., … Moshfeghm A. (2012). Sodium and potassium intakes among US adults. The American Journal of Clinical Nutrition, 96(3), 647-657.
Dhondup, T., & Qian, Q. (2017). Electrolyte and acid-base disorders in chronic kidney disease and end-stage kidney failure. Blood Purification, 43(1-3), 179-188.
Falkner (2017). Does potassium deficiency contribute to hypertension in children and adolescents? Current Hypertension Reports, 19(5), 1-6.
Garrison, S. R., Allan, G. M., Sekhon, R. K., Musini, V. M., & Khan, K. M. (2012). Magnesium for skeletal muscle cramps. Cochrane Database for Systematic Reviews, 9(9), 1-51.
Gennari (1998). Hypokalemia. The New England Journal of Medicine, 9(9), 1-51.
Gropper, S. S., Smith, J. L., & Carr, T. P. (2018). Advanced nutrition and human metabolism (7thed.). Boston, MA: Cengage Learning.
Han, P., Trinidad, B. J., & Shi, J. (2015). Hypocalcemia-induced seizure: Demystifying the paradox.American Society for Neurochemistry, 7(2), 1-9.
Jankovic, S. (2018). Treatment of hypokalemia. Racionalna Terapija, 7(1), 19-22.
Kenney, W. L., Wilmore, J. H., & Costill, D. L. (2012). Physiology of sport and exercise(5thed.). Champaign, IL: Human Kinetics.
Kovesdy, C. P., Appel, L. J., Grams, M. E., Gutekunst, L., McCullough, P. A., Palmer, B., F., … Townsend, R. R. (2017). Potassium homeostasis in health and disease: A scientific workshop cosponsored by the national kidney foundation and the American society of hypertension, 70(6), 844-858.
Lanham-New, S. A., Lambert, H., & Frasetto, L. (2012). Potassium.Advances in Nutrition, 3(6), 820-821.
Lecube, A., Baena-Fustegueras, J. A., Fort, J. M., Pelegri, D., Hernandez, C., & Simo R. (2012) (2012). Diabetes is a main factor accounting for hypomagnesemia in obese subjects.PLOS One, 7(1), 1-7.
Mao, I. F., Chen, M. L., & Ko, Y. C. (2001). Electrolyte loss in sweat and iodine deficiency in a hot environment. Archives of Environmental Health, 56(3), 271-277.
Popovic, Z., Momenroodaki, P., & Scheeler, R. (2014). Toward wearable wireless thermometers for internal body temperature measurements. IEEE Communications Magazine, 52(10), 118-125.
Potter, J. D., Robertson, S. P., & Johnson, J. D. (1981). Magnesium and the regulation of muscle contraction. Federation Proceedings, 40(12), 2653-2656.
Reisner, E. G., & Reisner, H. M. (2017). An introduction to human disease: Pathology and pathophysiology correlations (10thed.). Burlington, MA: Jones & Bartlett Learning.
Schwalfenberg, G. K., & Genuis, S. J. (2017). The importance of magnesium in clinical healthcare. Scientifica, 2017, 1-14.
Seyama, I., & Irisawa, H. (1967). The effect of high sodium concentration on the action potential of the skate heart. The Journal of General Physiology, 50(3), 505-517.
Wilcock, A., & Twycross, R.(2013). Magnesium. Journal of Pain and Symptom Management, 45(1), 137-144.
-Michael McIsaac